Magnetic bearing: structure, model, and control strategy
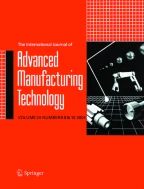
Bearings are pivotal components in mechanical systems, providing crucial support to rotating bodies. However, traditional bearings are susceptible to failure caused by friction and wear. This vulnerability is particularly pronounced in scenarios involving ultrahigh speeds and extreme conditions, necessitating the minimization of bearing losses and the enhancement of performance. Magnetic bearings, distinguished by their frictionless operation, absence of lubrication requirements, and high-speed capabilities, offer a promising solution to mitigate bearing failure attributable to friction. Nevertheless, a comprehensive review of magnetic bearings, encompassing their structural attributes, modeling mechanisms, and control strategies, is currently lacking in the literature. This paper aims to address this gap by conducting an exhaustive literature review on magnetic bearings. The objective is to provide scientists with a profound understanding of the structural characteristics, operational mechanisms, control performance, and future development trajectories of this technology. The paper begins by categorizing various magnetic bearings and conducting an in-depth analysis of their properties and characteristics, focusing on their magnetic circuit structures. Subsequently, it delves into the working principles and performance of mathematical models for magnetic bearings with different configurations, outlining the modeling procedures and optimization approaches. Additionally, the paper highlights the impact of control strategies on the performance of magnetic bearings. Modern control theory has demonstrated a remarkable 50% improvement in position accuracy and adjustment time compared to traditional PID control. Finally, the paper offers a glimpse into the future of magnetic bearing design, modeling mechanisms, and control strategies, presenting prospective directions for further advancements in this field.
This is a preview of subscription content, log in via an institution to check access.
Access this article
Subscribe and save
Springer+ Basic
€32.70 /Month
- Get 10 units per month
- Download Article/Chapter or eBook
- 1 Unit = 1 Article or 1 Chapter
- Cancel anytime
Buy Now
Price includes VAT (France)
Instant access to the full article PDF.
Rent this article via DeepDyve
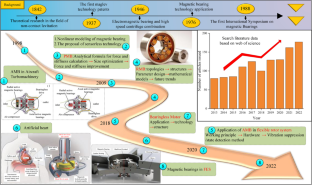
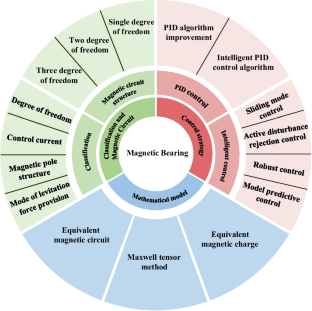
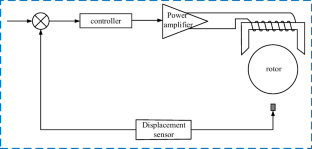
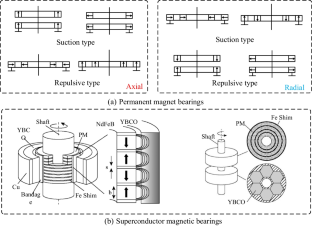
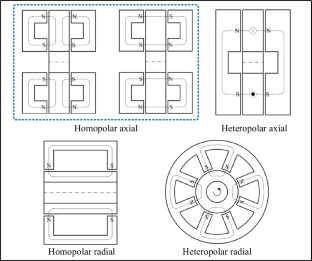
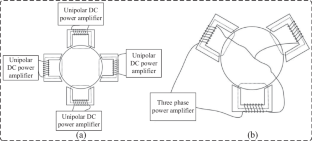
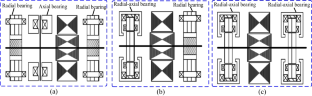
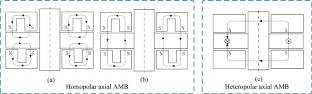
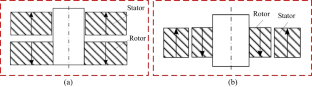
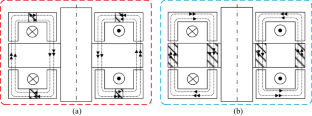
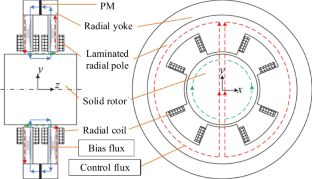
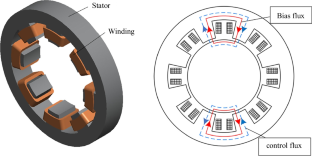
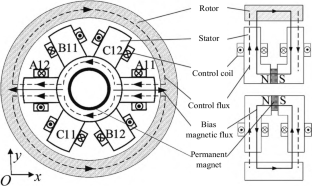
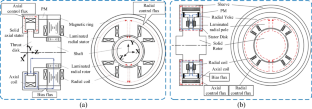
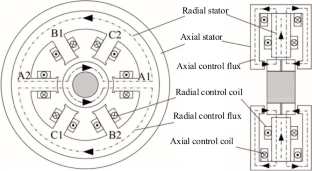

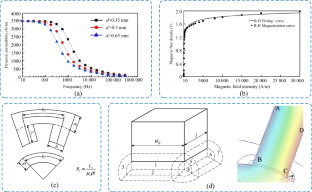
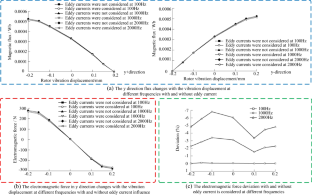
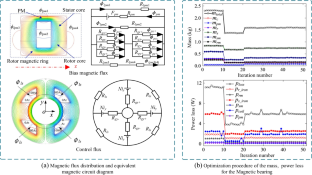

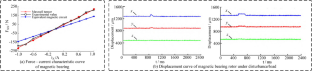
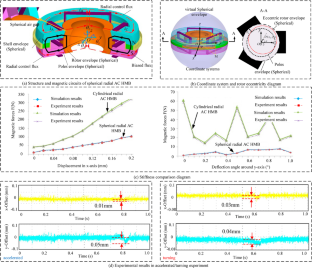

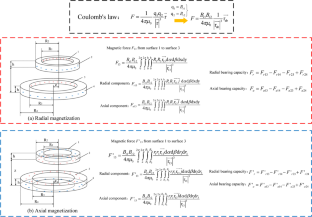
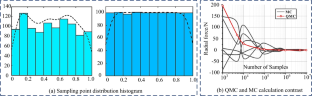
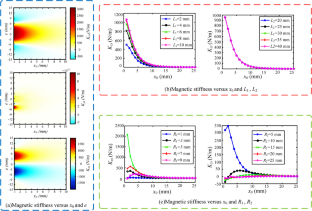
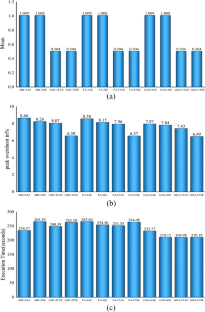
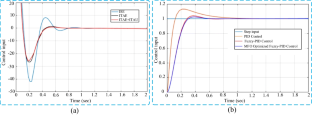
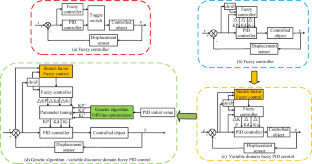
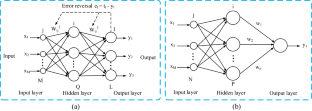
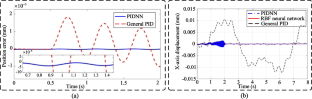
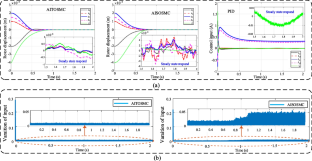
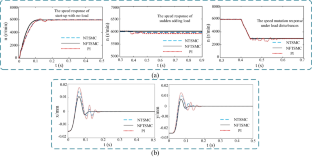

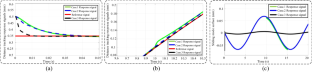
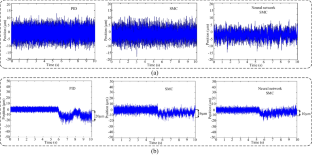
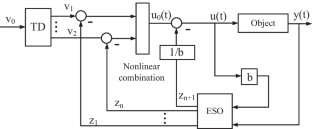
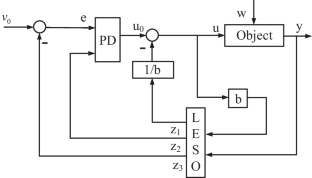
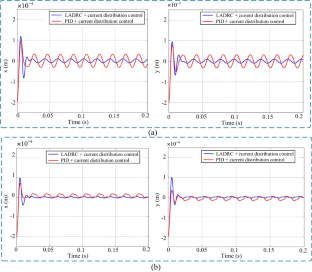
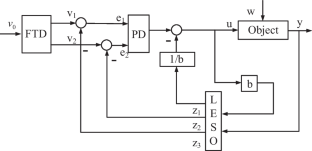
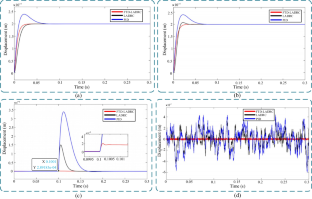
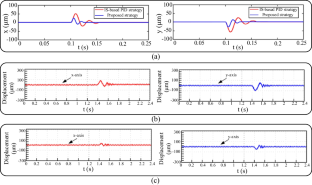
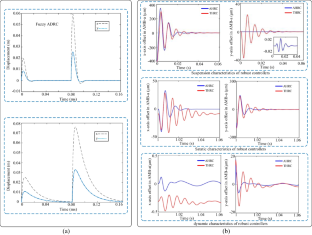
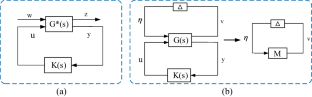
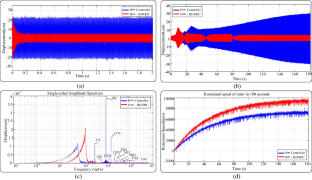
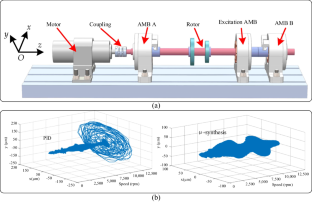
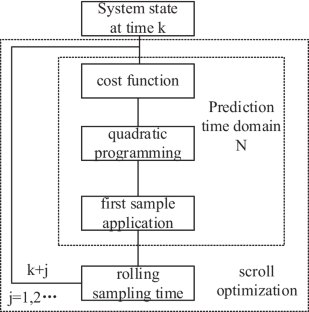
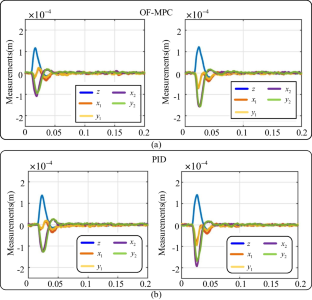
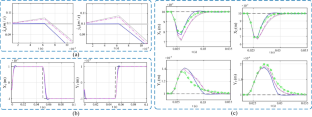
Similar content being viewed by others
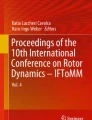
Progress in Calibrating Active Magnetic Bearings with Numerical and Experimental Approaches
Chapter © 2019
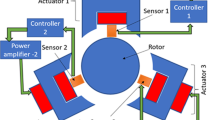
Design and speed control of U-type 3-coil active magnetic bearing
Article 29 April 2023
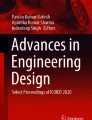
Design of Hybrid Bearings and Its Development: A Review
Chapter © 2021
Data availability
Data sharing is not applicable to this article.
Code availability
Code availability is not applicable to this article.
Abbreviations
Active magnetic bearing
Passive magnetic bearing
Hybrid magnetic bearing
Degrees of freedom
Equivalent magnetic circuit method
Monte Carlo method
Active magnetic bearing high-speed flywheel rotor system
Cuckoo search algorithm
Grasshopper optimization algorithm
Artificial bee colony algorithm
Extended state observer
Linear parameter-varying model predictive control
Integration time times absolute error
Integration time times squared error
Moth flame optimization
Radial basis function network
Terminal sliding mode control
Fast terminal sliding mode control
Nonsingular terminal sliding mode control method
Nonsingular terminal sliding mode control
Nonsingular fast terminal sliding mode control strategy
Adaptive second-order nonsingular fast terminal sliding mode control
Active disturbance rejection control
Linear active disturbance rejection controller
Linear active disturbance rejection controller
Offset-free model predictive control
Finite element calculation
References
- Sun XD, Chen L, Yang ZB (2013) Overview of bearingless permanent-magnet synchronous motors. IEEE T Ind Electron 60:5528–5538. https://doi.org/10.1109/tie.2012.2232253ArticleGoogle Scholar
- Samanta P, Hirani H (2008) Magnetic bearing configurations: theoretical and experimental studies. IEEE Trans Magn 44:292–300. https://doi.org/10.1109/tmag.2007.912854ArticleGoogle Scholar
- Andriollo M, Fanton E, Tortella A (2023) A review of innovative electromagnetic technologies for a totally artificial heart. Appl Sci 13:1870. https://doi.org/10.3390/app13031870ArticleCASGoogle Scholar
- Rogers JG, Pagani FD, Tatooles AJ, Bhat G, Slaughter MS, Birks EJ, Boyce SW, Najjar SS, Jeevanandam V, Anderson AS, Gregoric ID, Mallidi H, Leadley K, Aaronson KD, Frazier OH, Milano CA (2017) Intrapericardial left ventricular assist device for advanced heart failure. N Engl J Med 376:451–460. https://doi.org/10.1056/NEJMoa1602954ArticlePubMedGoogle Scholar
- Li XJ, Palazzolo A, Wang ZY (2021) A combination 5-DOF active magnetic bearing for energy storage flywheels. IEEE Trans Transp Electrification 7:2344–2355. https://doi.org/10.1109/tte.2021.3079402ArticleGoogle Scholar
- Earnshaw S (1848) On the nature of the molecular forces which regulate the constitution of the luminiferous ether. Trans Camb Philos Soc 7:97 Google Scholar
- Kemper H (1937) Overhead suspension railway with wheel-less vehicles employing magnetic suspension from iron rails. Germ Pat 1937:643316–644302 Google Scholar
- Beams J, Young J III, Moore J (1946) The production of high centrifugal fields. J Appl Phys 17:886–890. https://doi.org/10.1063/1.1707658ArticleGoogle Scholar
- Ji JC, Hansen CH, Zander AC (2008) Nonlinear dynamics of magnetic bearing systems. J Intel Mat Syst Str 19:1471–1491. https://doi.org/10.1177/1045389x08088666ArticleGoogle Scholar
- Ravaud R, Lemarquand G, Lemarquand V (2009) Force and stiffness of passive magnetic bearings using permanent magnets. Part 2: radial magnetization. IEEE Trans Magn 45:3334–3342. https://doi.org/10.1109/tmag.2009.2025315ArticleCASGoogle Scholar
- Ravaud R, Lemarquand G, Lemarquand V (2009) Force and stiffness of passive magnetic bearings using permanent magnets. Part 1: axial magnetization. IEEE Trans Magn 45:2996–3002. https://doi.org/10.1109/tmag.2009.2016088ArticleCASGoogle Scholar
- Zhang WY, Zhu HQ (2017) Radial magnetic bearings: an overview. Results Phys 7:3756–3766. https://doi.org/10.1016/j.rinp.2017.08.043ArticleGoogle Scholar
- Srinivas RS, Tiwari R, Kannababu C (2018) Application of active magnetic bearings in flexible rotordynamic systems—a state-of-the-art review. Mech Syst Signal Pr 106:537–572. https://doi.org/10.1016/j.ymssp.2018.01.010ArticleGoogle Scholar
- Li XJ, Palazzolo A (2022) A review of flywheel energy storage systems: state of the art and opportunities. J Energy Storage 46:103576. https://doi.org/10.1016/j.est.2021.103576
- Olabi AG, Wilberforce T, Abdelkareem MA, Ramadan M (2021) Critical review of flywheel energy storage system. Energies 14:2159. https://doi.org/10.3390/en14082159ArticleCASGoogle Scholar
- Zhang XT, Li CH, Zhou ZM, Liu B, Zhang YB, Yang M, Gao T, Liu MZ, Zhang NQ, Said Z, Sharma S, Ali HM (2023) Vegetable oil-based nanolubricants in machining: from physicochemical properties to application. Chin J Mech Eng 36:76. https://doi.org/10.1186/s10033-023-00895-5ArticleCASGoogle Scholar
- Liuyang Li, Yanbin Z, Cui X, Said Z, Sharma S, Liu M, Gao T, Zhou Z, Wang X, Li C (2023) Mechanical behavior and modeling of grinding force: a comparative analysis. J Manuf Process. https://doi.org/10.1016/j.jmapro.2023.07.074ArticleGoogle Scholar
- Dambatta YS, Li CH, Yang M, Beikai LI, Gao T, Liu MZ, Cui X, Wang XM, Zhang YB, Said Z, Sharma S, Zhou ZM (2023) Grinding with minimum quantity lubrication: a comparative assessment. Int J Adv Manuf Technol 128:955–1014. https://doi.org/10.1007/s00170-023-11962-5ArticleGoogle Scholar
- Yang M, Kong M, LI C, Long Y, Zhang Y, Sharma S, Li R, Gao T, Liu M, Cui X (2023) Temperature field model in surface grinding: a comparative assessment. Int J Extreme Manuf 5:042011. https://doi.org/10.1088/2631-7990/acf4d4
- Wang XM, Song YX, Li CH, Zhang YB, Ali HM, Sharma S, Li RZ, Yang M, Gao T, Liu MZ, Cui X, Said Z, Zhou ZM (2023) Nanofluids application in machining: a comprehensive review. Int J Adv Manuf Technol. https://doi.org/10.1007/s00170-022-10767-2ArticlePubMedPubMed CentralGoogle Scholar
- Koehler BU, Denk J, Van Maanen G, Lang M (2017) Applying standard industrial components for active magnetic bearings. Actuators 6:8. https://doi.org/10.3390/act6010008ArticleGoogle Scholar
- Denk J (2015) Active magnetic bearing technology running successfully in Europe’s biggest onshore gas field. Oil Gas-Eur Mag 41:91–92 Google Scholar
- Ye XT, Le QY, Zhou ZW (2020) A novel homopolar four degrees of freedom hybrid magnetic bearing. IEEE Trans Magn 56:6703404. https://doi.org/10.1109/tmag.2020.3001935ArticleGoogle Scholar
- Soni T, Dutt JK, Das AS (2019) Parametric stability analysis of active magnetic bearing supported rotor system with a novel control law subject to periodic base motion. IEEE T Ind Electron 67:1160–1170. https://doi.org/10.1109/TIE.2019.2898604ArticleGoogle Scholar
- Fonseca CA, Santos I, Weber HI (2020) An experimental and theoretical approach of a pinned and a conventional ball bearing for active magnetic bearings. Mech Syst Signal Pr 138:106541. https://doi.org/10.1016/j.ymssp.2019.106541
- Huang T, Zheng M, Zhang G (2019) A review of active magnetic bearing control technology. In: 2019 Chinese Control And Decision Conference (CCDC), pp 2888–2893. https://doi.org/10.1109/CCDC.2019.8833062
- JC H, HC W, KP F, Q L Overview of permanent magnet bearing structure. Bearing 7:1–7. https://doi.org/10.19533/j.issn1000-3762.2023.07.001
- Bachovchin KD, Hoburg JF, Post RF (2013) Stable levitation of a passive magnetic bearing. IEEE Trans Magn 49:609–617. https://doi.org/10.1109/tmag.2012.2209123ArticleGoogle Scholar
- Bekinal SI, Deshwal D, Srinivas VG (2022) Optimized multi-layer radial permanent magnet bearing with an eddy current damping systems. J Braz Soc Mech Sci 44:542. https://doi.org/10.1007/s40430-022-03855-7ArticleGoogle Scholar
- Karmakarand M, Sarkar S (2022) Semi-analytical finite element approach for 6-DOF characterizations of PMB Load. IEEE Trans Magn 58:8001510. https://doi.org/10.1109/tmag.2022.3174449ArticleGoogle Scholar
- Komori M, Yamanaka K, Asami K, Sakai N (2018) Proposal of new superconducting magnetic bearing using high T-c superconducting bulk and coil. IEEE Trans Magn 54:8300304. https://doi.org/10.1109/tmag.2018.2841943ArticleCASGoogle Scholar
- Johnson BR, Columbro F, Araujo D, Limon M, Smiley B, Jones G, Reichborn-Kjennerud B, Miller A, Gupta S (2017) A large-diameter hollow-shaft cryogenic motor based on a superconducting magnetic bearing for millimeter-wave polarimetry. Rev Sci Instrum 88:105102. https://doi.org/10.1063/1.4990884
- Mukoyama S, Nakao K, Sakamoto H, Matsuoka T, Nagashima K, Ogata M, Yamashita T, Miyazaki Y, Miyazaki K, Maeda T, Shimizu H (2017) Development of superconducting magnetic bearing for 300 kW flywheel energy storage system. IEEE T Appl Supercon 27:3600804. https://doi.org/10.1109/tasc.2017.2652327ArticleGoogle Scholar
- Han BC, He Z, Wen T, Zheng SQ (2023) Design, optimization and test of RHMB considering dynamic characteristics. Int J Mech Sci 238:107847. https://doi.org/10.1016/j.ijmecsci.2022.107847
- Li QH, Wang WM, Chu FL (2019) Damping ratio identification in rotordynamic system with inverse directional Fourier transform, multiple-tests-multiple-outputs and clustering techniques. Int J Mech Sci 151:797–807. https://doi.org/10.1016/j.ijmecsci.2018.12.020ArticleGoogle Scholar
- Zhu RZ, Xu W, Ye CY, Zhu JG, Lei G, Li X (2018) Design optimization of a novel heteropolar radial hybrid magnetic bearing using magnetic circuit model. IEEE Trans Magn 54:8201105. https://doi.org/10.1109/tmag.2017.2749759ArticleGoogle Scholar
- Zhou JX, Zheng SQ, Han BC, Fang JC (2017) Effects of notch filters on imbalance rejection with heteropolar and homopolar magnetic bearings in a 30-kW 60 000-r/min Motor. IEEE T Ind Electron 64:8033–8041. https://doi.org/10.1109/tie.2017.2694412ArticleGoogle Scholar
- Kurnyta-Mazurek P, Kurnyta A, Henzel M (2020) Measurement system of a magnetic suspension system for a jet engine rotor. Sensors 20:862. https://doi.org/10.3390/s20030862ArticlePubMedPubMed CentralGoogle Scholar
- L C, X ZY, W CX, W YJ (2022) Research on non-directional differential control strategy of active magnetic bearing. J Mech Eng 58:161–170. https://doi.org/10.3901/JME.2022.21.161ArticleGoogle Scholar
- Z HQ, Z RZ (2014) Technology research progress of ac magnetic bearings. Electr Mach Control Appl 41:45–50 Google Scholar
- Darbandi SM, Behzad M, Salarieh H, Mehdigholi H (2014) Linear output feedback control of a three-pole magnetic bearing. IEEE-Asme T Mech 19:1323–1330. https://doi.org/10.1109/tmech.2013.2280594ArticleGoogle Scholar
- Ju JT, Zhu HQ (2016) Radial force-current characteristics analysis of three-pole radial-axial hybrid magnetic bearings and their structure improvement. Energies 9:706. https://doi.org/10.3390/en9090706ArticleGoogle Scholar
- Zhu HQ, Ding SL, Jv JT (2016) Modeling for three-pole radial hybrid magnetic bearing considering edge effect. Energies 9:345. https://doi.org/10.3390/en9050345ArticleGoogle Scholar
- Wu MY, Zhu HQ (2023) Electromagnetic characteristics and capacity analysis of a radial-axial hybrid magnetic bearing with two different radial stators. Electronics 12:1493. https://doi.org/10.3390/electronics12061493ArticleGoogle Scholar
- Ahn J, Han C, Kim C, Park C, Choi J (2017) Design of hybrid thrust magnetic bearing for heavy rotating shaft considering self-weight compensation according to axial load. In: 2017 IEEE International Magnetics Conference (INTERMAG), pp 1-1. https://doi.org/10.1109/INTMAG.2017.8007919
- Sun X, Jin Z, Chen L, Yang Z (2021) Disturbance rejection based on iterative learning control with extended state observer for a four-degree-of-freedom hybrid magnetic bearing system. Mech Syst Signal Pr 153:107465. https://doi.org/10.1016/j.ymssp.2020.107465ArticleGoogle Scholar
- Sun XD, Su BK, Chen L, Yang ZB, Xu X, Shi Z (2017) Precise control of a four degree-of-freedom permanent magnet biased active magnetic bearing system in a magnetically suspended direct-driven spindle using neural network inverse scheme. Mech Syst Signal Pr 88:36–48. https://doi.org/10.1016/j.ymssp.2016.11.022ArticleGoogle Scholar
- Brenkacz L, Witanowski L, Drosinska-Komor M, Szewczuk-Krypa N (2021) Research and applications of active bearings: a state-of-the-art review. Mech Syst Signal Pr 151:107423. https://doi.org/10.1016/j.ymssp.2020.107423
- Ye XT, Yan YM, Jia CL, Zhang T (2022) Modeling and design of a novel 5-DOF AC-DC hybrid magnetic bearing. Appl Sci-Basel 12:8931. https://doi.org/10.3390/app12188931ArticleCASGoogle Scholar
- Zhang WY, Zhu HQ, Yang HK, Chen T (2017) Experimental analysis and full prediction model of a 5-DOF motorized spindle. Energies 10:75. https://doi.org/10.3390/en10010075ArticleGoogle Scholar
- Zou WL, Wang XL, Deng ZQ, Liu SL (2015) Designing a single-side hybrid axial magnetic bearing based on bias/control magnetic field ratio restrictions. Mech Sci Technol Aerosp Eng 34:1536–1542. https://doi.org/10.13433/j.cnki.1003-8728.2015.1012ArticleGoogle Scholar
- Falkowski K (2012) The rings with molecular current as the model of the passive magnetic bearing. J Vibroengineering 14:135–142 Google Scholar
- Matuszewski L, Falkowski K (2010) Mathematical model of radial passive magnetic bearing. Pol Marit Res 17:37–44. https://doi.org/10.2478/v10012-010-0026-zArticleGoogle Scholar
- Sun JJ, Ren YA, Fang JC (2011) Passive axial magnetic bearing with Halbach magnetized array in magnetically suspended control moment gyro application. J Magn Magn Mater 323:2103–2107. https://doi.org/10.1016/j.jmmm.2011.02.020ArticleCASGoogle Scholar
- Supreeth DK, Bekinal SI, Chandranna SR (2022) An overview on electrodynamic bearings. IEEE Access 10:57437–57451. https://doi.org/10.1109/access.2022.3176632ArticleGoogle Scholar
- Oberbeck C, Ulbrich H. (2000) Active compensation of the eigen-dynamics of electromagnetic actuators by Ecu-based non-linear feedback control. In: Proceedings of the 7th International Symposium on Magnetic Bearings
- Yu CM, Deng ZQ, Chen SS, Mei L, Peng C, Cao X (2022) A novel subdomain and magnetic circuit modeling method for hybrid homopolar radial magnetic bearings. Mech Syst Signal Pr 170:108823. https://doi.org/10.1016/j.ymssp.2022.108823
- Yu CM, Deng ZQ, Mei L, Chen SS, Peng C, Ding Q (2021) Accurate modelling based on a subdomain method for heteropolar radial magnetic bearing with a solid rotor. IET Electr Power Appl 15:1232–1244. https://doi.org/10.1049/elp2.12091ArticleGoogle Scholar
- Matsuda K, Kanemitsu Y, Kijimoto S (2007) Optimal number of stator poles for compact active radial magnetic bearings. IEEE Trans Magn 43:3420–3427. https://doi.org/10.1109/tmag.2007.900184ArticleGoogle Scholar
- Grbesa B (1998) Low loss and low cost active radial homopolar magnetic bearing. Proc Sixth Int Symp Magn Bearings 286–295
- Chen S-L, Hsu C-T (2002) Optimal design of a three-pole active magnetic bearing. IEEE Trans Magn 38:3458–3466. https://doi.org/10.1109/TMAG.2002.802709ArticleGoogle Scholar
- Chen S-L, Chen S-H, Yan S-T (2005) Experimental validation of a current-controlled three-pole magnetic rotor-bearing system. IEEE Trans Magn 41:99–112. https://doi.org/10.1109/TMAG.2004.839735ArticleGoogle Scholar
- Pesch AH, Smirnov A, Pyrhonen O, Sawicki JT (2015) Magnetic bearing spindle tool tracking through mu-synthesis robust control. IEEE-ASME T Mech 20:1448–1457. https://doi.org/10.1109/tmech.2014.2344592ArticleGoogle Scholar
- Zhang WY, Zhu HQ (2015) Control system design for a five-degree-of-freedom electrospindle supported with AC hybrid magnetic bearings. IEEE-ASME T Mech 20:2525–2537. https://doi.org/10.1109/tmech.2014.2387151ArticleGoogle Scholar
- Zhang WY, Zhu HQ, Yang ZB, Sun XD, Yuan Y (2016) Nonlinear model analysis and “switching model” of AC-DC three-degree-of-freedom hybrid magnetic bearing. IEEE-ASME T Mech 21:1102–1115. https://doi.org/10.1109/tmech.2015.2463676ArticleGoogle Scholar
- Ji L, Xu LX, Jin CW (2013) Research on a low power consumption six-pole heteropolar hybrid magnetic bearing. IEEE Trans Magn 49:4918–4926. https://doi.org/10.1109/tmag.2013.2238678ArticleGoogle Scholar
- Morrison CR, Siebert MW, Ho EJ (2008) Electromagnetic forces in a hybrid magnetic-bearing switched-reluctance motor. IEEE Trans Magn 44:4626–4638. https://doi.org/10.1109/tmag.2008.2002891ArticleGoogle Scholar
- Noh M, Gruber W, Trumper DL (2017) Hysteresis bearingless slice motors with homopolar flux-biasing. IEEE-ASME T Mech 22:2308–2318. https://doi.org/10.1109/tmech.2017.2740429ArticleGoogle Scholar
- Wajnert D (2019) Analysis of the cross-coupling effect and magnetic force nonlinearity in the 6-pole radial hybrid magnetic bearing. Int J Appl Electrom 61:43–57. https://doi.org/10.3233/jae-180088ArticleGoogle Scholar
- Zhu HQ, Liu TT (2020) Rotor Displacement self-sensing modeling of six-pole radial hybrid magnetic bearing using improved particle swarm optimization support vector machine. IEEE T Power Electr 35:12296–12306. https://doi.org/10.1109/tpel.2020.2982746ArticleMathSciNetGoogle Scholar
- Yu CM, Deng ZQ, Mei L, Peng C, Cao X (2023) Multiobjective optimization of 3-DOF magnetic bearing considering eddy current effects and saturation. Mech Syst Signal Pr 182:109538. https://doi.org/10.1016/j.ymssp.2022.109538
- Le Y, Wang K (2016) Design and optimization method of magnetic bearing for high-speed motor considering Eddy current effects. IEEE-ASME T Mech 21:2061–2072. https://doi.org/10.1109/tmech.2016.2569822ArticleGoogle Scholar
- Ren XJ, Le Y, Sun JJ, Han BC, Wang K (2017) Magnetic flux leakage modelling and optimisation of a CRAHMB for DC motor. IET Electr Power Appl 11:212–221. https://doi.org/10.1049/iet-epa.2016.0259ArticleGoogle Scholar
- Zhong YL, Wu LJ, Huang XY, Fang YT (2019) Modeling and design of a 3-DOF magnetic bearing with toroidal radial control coils. IEEE Trans Magn 55:8300907. https://doi.org/10.1109/tmag.2019.2914510ArticleCASGoogle Scholar
- Ling X, Xiwu H, Wenjie C, Ming L (2022) Structural optimization and dynamic characteristics of the new type 3-degrees of freedom axial and radial hybrid magnetic bearing. P I Mech Eng C-J Mec 236:5097–5110. https://doi.org/10.1177/09544062211052826ArticleGoogle Scholar
- Wang SS, Zhu HQ, Wu MY, Zhang WY (2020) Active disturbance rejection decoupling control for three-degree-of- freedom six-pole active magnetic bearing based on BP neural network. IEEE T Appl Supercon 30:1–5. https://doi.org/10.1109/tasc.2020.2990794ArticleCASGoogle Scholar
- Zhu H, Wang S (2020) Decoupling control based on linear/non-linear active disturbance rejection switching for three-degree-of-freedom six-pole active magnetic bearing. Electr Power Appl IET 14:1818–1827. https://doi.org/10.1049/iet-epa.2019.0448ArticleGoogle Scholar
- Abdollha MB, Tokhi MO (2016) Nonlinear full-order observer-based controller design for active magnetic levitation via LQR-feedback linearisation. Int J Model Ident Control 26:59–67 ArticleGoogle Scholar
- Zhang WY, Zhu HQ, Yuan Y (2015) Study on key technologies and applications of magnetic bearings. Trans China Electrotechnical Soc 30:12–20. https://doi.org/10.19595/j.cnki.1000-6753.tces.2015.12.002ArticleCASGoogle Scholar
- Zhang WY, Huangqiu Z, Ju JT, Chen T (2016) Research status and development of magnetic bearings. Bearing 12:56–63. https://doi.org/10.19533/j.issn1000-3762.2016.12.015ArticleCASGoogle Scholar
- Hao J, Li CY, Song WJ, Yao ZH, Miao HH, Xu MT, Gong XX, Lu H, Liu ZD (2023) Thermal-mechanical dynamic interaction in high-speed motorized spindle considering nonlinear vibration. Int J Mech Sci 240:107959. https://doi.org/10.1016/j.ijmecsci.2022.107959
- Wu C, Li SS (2023) Modeling, design and suspension force analysis of a novel AC six-pole heteropolar hybrid magnetic bearing. Appl Sci-Basel 13:1643. https://doi.org/10.3390/app13031643ArticleCASGoogle Scholar
- Zhang H, Zhu HQ, Wu MY (2022) Multi-objective parameter optimization-based design of six-pole radial hybrid magnetic bearing. IEEE J Em Sel Top P 10:4526–4535. https://doi.org/10.1109/jestpe.2021.3125488ArticleGoogle Scholar
- Zhou JY, Zhu HQ (2022) Development and research summary of magnetic bearings. Micromotors 55:93–98. https://doi.org/10.15934/j.cnki.micromotors.2022.06.003ArticleGoogle Scholar
- Fu J, Wang XG, Hou LL, Pan A (2018) Structure study and performance analysis on the new laminated core type axial redundant magnetic bearing. Int J Appl Electrom 58:101–120. https://doi.org/10.3233/jae-180008ArticleGoogle Scholar
- Le Y, Sun JJ, Han BC (2016) Modeling and design of 3-DOF magnetic bearing for high-speed motor including eddy-current effects and leakage effects. IEEE T Ind Electron 63:3656–3665. https://doi.org/10.1109/tie.2016.2530778ArticleGoogle Scholar
- Zhang PF, Wang ZH, Xi G (2019) A multi-objective optimal design methodology for solid core no-thrust-disk/thrust hybrid magnetic bearings considering eddy-current effects and leakage. Int J Appl Electrom 61:13–42. https://doi.org/10.3233/jae-180062ArticleGoogle Scholar
- Seifert R, Robenack K, Hofmann W (2019) Rational approximation of the analytical model of nonlaminated cylindrical magnetic actuators for flux estimation and control. IEEE Trans Magn 55:8301016. https://doi.org/10.1109/tmag.2019.2936791ArticleGoogle Scholar
- Zhu YS, Yang DS, Wang X, Hu B, Zhang PJ (2022) Research on fractional-order modeling of nonlaminated electromagnetic bearings considering eddy current effects. IEEE Trans Magn 58:8300208. https://doi.org/10.1109/tmag.2021.3134012ArticleGoogle Scholar
- Wang DX, Wang NX, Ye C, Chen KS (2017) Research on analytical bearing capacity model of active magnetic bearings based on magnetic saturation. IET Electr Power Appl 11:1548–1557. https://doi.org/10.1049/iet-epa.2017.0106ArticleGoogle Scholar
- Romanenko A, Smirnov A, Jastrzebski RP, Pyrhönen O (2014) Losses estimation and modelling in active magnetic bearings. In: 2014 16th European Conference on Power Electronics and Applications, pp 1–8. https://doi.org/10.1109/EPE.2014.6910753
- Pinzhou Y, Hongwei L, Jing T, Wentao Y (2019) Influence of rotor vibration on magnetic field distribution of radial active magnetic bearings. In: 2019 14th IEEE Conference on Industrial Electronics and Applications (ICIEA) pp 2399–2404. https://doi.org/10.1109/ICIEA.2019.8833941
- Asami K, Chiba A, Rahman MA, Hoshino T, Nakajima A (2005) Stiffness analysis of a magnetically suspended bearingless motor with permanent magnet passive positioning. IEEE Trans Magn 41:3820–3822. https://doi.org/10.1109/TMAG.2005.854933ArticleGoogle Scholar
- Chiba A, Kiryu K, Rahman MA, Fukao T (2006) Radial force and speed detection for improved magnetic suspension in bearingless motors. IEEE Trans Ind Appl 42:415–422. https://doi.org/10.1109/TIA.2005.863911ArticleGoogle Scholar
- Zhang T, Liu XF, Mo LH, Ye XT, Ni W, Ding WH, Huang J, Wang X (2018) Modeling and analysis of hybrid permanent magnet type bearingless motor. IEEE Trans Magn 54:8102804. https://doi.org/10.1109/tmag.2017.2766661ArticleGoogle Scholar
- Zhang WY, Zhu HQ (2013) Precision modeling method specifically for AC magnetic bearings. IEEE Trans Magn 49:5543–5553. https://doi.org/10.1109/tmag.2013.2252358ArticleGoogle Scholar
- Ma ZH, Liu G, Liu YC, Yang ZC, Zhu HQ (2022) Research of a six-pole active magnetic bearing system based on a fuzzy active controller. Electronics 11:1723. https://doi.org/10.3390/electronics11111723ArticleGoogle Scholar
- Zhu HQ, F S (2021) Soft-sensing modeling for rotor displacements of six-pole radial active magnetic bearing using improved continuous hidden Markov model. Proc CSEE 41:3933–3943. https://doi.org/10.13334/j.0258-8013.pcsee.201007ArticleGoogle Scholar
- L G, Zhu HQ (2022) Calculation model for suspension force of six-pole outer-rotor hybrid magnetic bearing based on maxwell tensor method. Bearing 12:23–27. https://doi.org/10.19533/j.issn1000-3762.2022.12.004
- Liu G, Zhu HQ (2022) Displacement estimation of six-pole hybrid magnetic bearing using modified particle swarm optimization support vector machine. Energies 15:1610. https://doi.org/10.3390/en15051610ArticleCASGoogle Scholar
- Zhang WY, Wang Z (2023) Accurate modeling and control system design for a spherical radial AC HMB for a flywheel battery system. Energies 16:1973. https://doi.org/10.3390/en16041973ArticleCASGoogle Scholar
- Qu CA, Pei YC, Li ZX (2021) A study on magnetic force characteristics between two cuboidal permanent magnets. J Supercond Novel Magn 34:2441–2454. https://doi.org/10.1007/s10948-021-05927-6ArticleCASGoogle Scholar
- Janssen JL, Paulides JJ, Compter JC, Lomonova EA (2010) Three-dimensional analytical calculation of the torque between permanent magnets in magnetic bearings. IEEE Trans Magn 46:1748–1751. https://doi.org/10.1109/TMAG.2010.2043224ArticleGoogle Scholar
- Du Y, Ye C, Deng C, Yu D, Xiong F, Zhang J (2020) Calculation and verification of magnetic force in a radial permanent magnetic bearing based on quasi-Monte Carlo method. 2020 IEEE 1st China Int Youth Conf Electr Eng (CIYCEE) 1–5. https://doi.org/10.1109/CIYCEE49808.2020.9332684
- Yonnet J-P (1981) Permanent magnet bearings and couplings. IEEE Trans Magn 17:1169–1173. https://doi.org/10.1109/TMAG.1981.1061166ArticleGoogle Scholar
- Han BC, Zheng SQ, Le Y, Xu S (2013) Modeling and analysis of coupling performance between passive magnetic bearing and hybrid magnetic radial bearing for magnetically suspended flywheel. IEEE Trans Magn 49:5356–5370. https://doi.org/10.1109/tmag.2013.2263284ArticleGoogle Scholar
- Bachovchin KD, Hoburg JF, Post RF (2012) Magnetic fields and forces in permanent magnet levitated bearings. IEEE Trans Magn 48:2112–2120. https://doi.org/10.1109/tmag.2012.2188140ArticleGoogle Scholar
- Muscia R (2017) Magneto-mechanical model of passive magnetic axial bearings versus the eccentricity error, Part I: physical mathematical model. Appl Comput Electromagn Soc J 32:670–677 Google Scholar
- Z RY, Zhu CS (2019) Analysis and control of radial disturbing force of the axial permanent magnetic bearing in flywheel. J Mech Electr Eng 36:879–885. https://doi.org/10.3969/j.issn.1001-4551.2019.09.001ArticleMathSciNetGoogle Scholar
- Santra T, Roy D, Choudhury A, Yamada S (2019) Experimental verification of force and stiffness between two ring magnets calculated by Monte Carlo integration technique. J Inst Eng (India): Ser B 100:123–129. https://doi.org/10.1007/s40031-019-00373-4ArticleGoogle Scholar
- Zhang L, Wu HC, Li P, Hu YF, Song CS (2019) Design, analysis, and experiment of multiring permanent magnet bearings by means of equally distributed sequences based Monte Carlo method. Math Probl Eng 2019. https://doi.org/10.1155/2019/4265698
- Qu C, Pei YC, Yang F, Li ZX, Xin QY (2022) An investigation on the improved magnetic stiffness model and characteristic analysis for two cylindrical permanent magnets. Meccanica 57:677–696. https://doi.org/10.1007/s11012-021-01461-wArticleMathSciNetGoogle Scholar
- Yu W, Li X (2014) A magnetic levitation system for advanced control education. IFAC Proc 47:9032–9037. https://doi.org/10.3182/20140824-6-za-1003.00147ArticleGoogle Scholar
- Alves P (1998) Magnetic Bearings-A Primer. Proc 27th Turbomach Symp
- Chen M, Knospe CR (2007) Control approaches to the suppression of machining chatter using active magnetic bearings. IEEE Trans Control Syst Technol 15:220–232. https://doi.org/10.1109/TCST.2006.886419ArticleGoogle Scholar
- Basaran S, Sivrioglu S (2019) Novel repulsive magnetic bearing flywheel system with composite adaptive control. IET Electr Power Appl 13:676–685. https://doi.org/10.1049/iet-epa.2018.5312ArticleGoogle Scholar
- Wei C (2022) Survey of magnetic bearing control. Electr Eng 20:33–35. https://doi.org/10.19768/j.cnki.dgjs.2022.20.011ArticleGoogle Scholar
- Xu PS, Yang DS, Xu HS, Luo ZW (2021) A review of control method of magnetic bearing system. China Autom Congr 2021:283–288. https://doi.org/10.26914/c.cnkihy.2021.053518
- Tavakoli S, Safaei M (2018) Analytical PID control design in time domain with performance-robustness trade-off. Electron Lett 54:815–817. https://doi.org/10.1016/j.automatica.2019.06.017ArticleGoogle Scholar
- Beerens R, Bisoffi A, Zaccarian L, Heemels W, Nijmeijer H, van de Wouw N (2019) Reset integral control for improved settling of PID-based motion systems with friction. Automatica 107:483–492. https://doi.org/10.1016/j.automatica.2019.06.017ArticleMathSciNetGoogle Scholar
- Khan O, Madhuranthakam CMR, Douglas P, Lau H, Sun J, Farrell P (2018) Optimized PID controller for an industrial biological fermentation process. J Process Control 71:75–89. https://doi.org/10.1016/j.jprocont.2018.09.007ArticleCASGoogle Scholar
- Wu HC, Yu HT, Hu S, Chen P (2018) Analysis on characteristics of homo-polar permanent biased radial magnetic bearings. Bearing 7:40–45. https://doi.org/10.19533/j.issn1000-3762.2018.07.010
- Wang DX, Wang NX, Chen KS, Ye C (2019) Dynamic characteristics of magnetic suspended dual-rotor system by riccati transfer matrix method. Shock Vib 2019:9843732. https://doi.org/10.1155/2019/9843732ArticleGoogle Scholar
- Guan XD, Zhou J, Wu HT, Zhang Y (2019) Trajectory tracking of rotating shaft with active magnetic bearings under different reference signals. Appl Comput Electromagn Soc J 34:1435–1444 Google Scholar
- Kato H, Komori M, Asami K, Sakai N (2020) Development of one-axis active controlled bearingless motor working at extremely low temperature. J Adv Mech Des Syst 14:19–00337. https://doi.org/10.1299/jamdsm.2020jamdsm0034ArticleGoogle Scholar
- Soni T, Dutt JK, Das AS (2020) Parametric stability analysis of active magnetic bearing supported rotor system with a novel control law subject to periodic base motion. IEEE T Ind Electron 67:1160–1170. https://doi.org/10.1109/tie.2019.2898604ArticleGoogle Scholar
- Sarmah N, Tiwari R (2022) Numerical and experimental study on quantitative assessment of multiple fault parameters in a warped internally damped rotor with a transverse fatigue crack integrated with an active magnetic bearing. Mech Syst Signal Pr 174:109112. https://doi.org/10.1016/j.ymssp.2022.109112
- Majumder G, Tiwari R (2022) Experimental study on vibration control of spur geared rotor system with active magnetic bearings. J Sound Vib 532:117005. https://doi.org/10.1016/j.jsv.2022.117005
- Hutterer M, Wimmer D, Schrodl M (2020) Stabilization of a magnetically levitated rotor in the case of a defective radial actuator. IEEE/ASME Trans Mechatron 25:2599–2609. https://doi.org/10.1109/tmech.2020.2985623ArticleGoogle Scholar
- Chen LL, Zhu CS, Zhong ZX, Wang CK, Li ZN (2020) Radial position control for magnetically suspended high-speed flywheel energy storage system with inverse system method and extended 2-DOF PID controller. IET Electr Power Appl 14:71–81. https://doi.org/10.1049/iet-epa.2019.0512ArticleGoogle Scholar
- Wu HC, Yu MY, Song CS, Wang NX (2022) Unbalance vibration suppression of maglev high-speed motor based on the least-mean-square. Actuators 11:348. https://doi.org/10.3390/act11120348ArticleGoogle Scholar
- Laldingliana J, Biswas PK (2022) Artificial intelligence based fractional order PID control strategy for active magnetic bearing. J Electr Eng Technol 17:3389–3398. https://doi.org/10.1007/s42835-022-01102-6ArticleGoogle Scholar
- Gupta S, Biswas PK, Debnath S, Ghosh A, Babu TS, Zawbaa HM, Kamel S (2023) Metaheuristic optimization techniques used in controlling of an active magnetic bearing system for high-speed machining application. IEEE Access 11:12100–12118. https://doi.org/10.1109/access.2023.3241854ArticleGoogle Scholar
- Gupta S, Debnath S, Biswas PK (2023) Control of an active magnetic bearing system using swarm intelligence-based optimization techniques. Electr Eng 105:935–952. https://doi.org/10.1007/s00202-022-01707-0ArticleGoogle Scholar
- Abdelaty BG, Ahmed AH, Ouda AN (2018) Fixed set point weighting 2DOF PID controller for control processes. J Eng Math 2:21–27. https://doi.org/10.11648/j.engmath.20180201.13ArticleGoogle Scholar
- Liu CZ, Deng ZQ, Xie L, Li KX (2015) The design of the simple structure-specified controller of magnetic bearings for the high-speed SRM. IEEE-ASME T Mech 20:1798–1808. https://doi.org/10.1109/tmech.2014.2354985ArticleGoogle Scholar
- J Z, Zy X, Hj Y, X W (2015) Research of fuzzy control strategy for motorized spindle supported by active magnetic bearings. Mach Tool Hydraul 43:1–6. https://doi.org/10.3969/j.issn.1001-3881.2015.17.001ArticleGoogle Scholar
- Zhang W (2010) A flywheel energy storage system suspended by active magnetic bearings with fuzzy PID controller. ICCASM 2010 - 2010 Int Conf Comput Appl Syst Model Proc 5:5116–5119. https://doi.org/10.1109/iccasm.2010.5619052ArticleGoogle Scholar
- Yuan GZLMY (2012) Variable universe fuzzy PID control strategy of permanent magnet biased axial magnetic bearing used in magnetic suspension wind power generator. Lect Notes Comput Sc 7506:44–55. https://doi.org/10.1007/978-3-642-33509-9_4ArticleGoogle Scholar
- Noshadi A, Shi J, Lee WS, Shi P, Kalam A (2016) Optimal PID-type fuzzy logic controller for a multi-input multi-output active magnetic bearing system. Neural Comput Appl 27:2031–2046. https://doi.org/10.1007/s00521-015-1996-7ArticleGoogle Scholar
- Dhyani A, Panda MK, Jha B (2018) Moth-flame optimization-based fuzzy-PID controller for optimal control of active magnetic bearing system. IJST-T Electr Eng 42:451–463. https://doi.org/10.1007/s40998-018-0077-1ArticleGoogle Scholar
- Ye YY, Hu JD (2020) Design of magnetic suspended rotor controller based on fuzzy-PID double mode control. Electr Eng 12:15–16,19. https://doi.org/10.19768/j.cnki.dgjs.2020.12.006
- Qiao WZ, Mizumoto M (1996) PID type fuzzy controller and parameters adaptive method. Fuzzy Sets Syst 78:23–35. https://doi.org/10.1016/0165-0114(95)00115-8ArticleMathSciNetGoogle Scholar
- Liu MZ, Li CH, Yang M, Gao T, Wang XM, Cui X, Zhang YB, Said Z, Sharma S (2023) Mechanism and enhanced grindability of cryogenic air combined with biolubricant grinding titanium alloy. Tribol Int 187:108704. https://doi.org/10.1016/j.triboint.2023.108704
- Chen KY, Tung PC, Tsai MT, Fan YH (2009) A self-tuning fuzzy PID-type controller design for unbalance compensation in an active magnetic bearing. Expert Syst Appl 36:8560–8570. https://doi.org/10.1016/j.eswa.2008.10.055ArticleGoogle Scholar
- Yao D, Wang JW, Liu Y (2015) Enhancing working performance of active magnetic bearings using improved fuzzy control and Kalman-LMS filter. J Intell Fuzzy Syst 29:1343–1353. https://doi.org/10.3233/ifs-141485ArticleMathSciNetGoogle Scholar
- S ZR, Z YB, W HF (2020) Variable universe fuzzy PID control based on genetic optimization for magnetic bearings. Mod Electron Tech 43:94–97. https://doi.org/10.16652/j.issn.1004-373x.2020.15.022ArticleGoogle Scholar
- Hussain S, Mokhtar M, Howe JM (2015) Sensor failure detection, identification, and accommodation using fully connected cascade neural network. IEEE T Ind Electron 62:1683–1692. https://doi.org/10.1109/tie.2014.2361600ArticleGoogle Scholar
- Cui G, Su B, Cai B (2019) The design of levitation controller based on BPNN with quantization factors for Maglev Yaw system of wind turnines. In: 2019 Chinese Automation Congress (CAC) pp 4645-4650. https://doi.org/10.1109/CAC48633.2019.8996322
- Li Z, Yang K, Zhang Y, Liu A, Yang F (2020) Improved active disturbance rejection control of permanent-magnet synchronous motor based on BP neural network. In: 2020 23rd international conference on electrical machines and systems (ICEMS), pp 2137–2141. https://doi.org/10.23919/ICEMS50442.2020.9290892
- Ghritlahre HK, Prasad RK (2018) Exergetic performance prediction of solar air heater using MLP, GRNN and RBF models of artificial neural network technique. J Environ Manag 223:566–575. https://doi.org/10.1016/j.jenvman.2018.06.033ArticleGoogle Scholar
- Hamdi H, Regaya CB, Zaafouri A (2019) Real-time study of a photovoltaic system with boost converter using the PSO-RBF neural network algorithms in a MyRio controller. Sol Energy 183:1–16. https://doi.org/10.1016/j.solener.2019.02.064ArticleGoogle Scholar
- La Rosa CL, De Castro FCC, De Castro MCF, Müller C, Ribeiro SM (2018) Cognitive radio signal classification based on subspace decomposition and RBF neural networks. Wirel Netw 24:821–831. https://doi.org/10.1007/s11276-016-1376-yArticleGoogle Scholar
- Tang JQ, Zhang M, Cui X, Sun JJ, Zhou XX (2023) Neural network sliding model control of radial translation for magnetically suspended rotor (MSR) in control moment gyro. Actuators 12:217. https://doi.org/10.3390/act12060217ArticleGoogle Scholar
- Cui X, Li CH, Yang M, Liu MZ, Gao T, Wang XM, Said Z, Sharma S, Zhang YB (2023) Enhanced grindability and mechanism in the magnetic traction nanolubricant grinding of Ti-6Al-4 V. Tribol Int 186:108603. https://doi.org/10.1016/j.triboint.2023.108603
- Chen SY, Lin FJ (2013) Decentralized PID neural network control for five degree-of-freedom active magnetic bearing. Eng Appl Artif Intel 26:962–973. https://doi.org/10.1016/j.engappai.2012.11.002ArticleGoogle Scholar
- Du XX, Sun YH (2020) Control of hybrid electromagnetic bearing and elastic foil gas bearing under deep learning. PLoS ONE 15:e0243107. https://doi.org/10.1371/journal.pone.0243107
- He HT, Liu YB, Ba LM (2022) A nonlinear dynamic model of flywheel energy storage systems based on alternative concept of back propagation neural networks. J Comput Nonlin Dyn 17:091006. https://doi.org/10.1115/1.4054681
- Tang JQ, Zhao XF, Wang Y, Cui X (2019) Adaptive neural network control for rotor’s stable suspension of Vernier-gimballing magnetically suspended flywheel. P I Mech Eng I-J Sys 233:1017–1029. https://doi.org/10.1177/0959651818813625ArticleGoogle Scholar
- Tang JQ, Ning MY, Cui X, Wei TK, Zhao XF (2021) PIDNN control for Vernier-gimballing magnetically suspended flywheel under nonlinear change of stiffness and disturbance. P I Mech Eng I-J Sys 235:1100–1112. https://doi.org/10.1177/0959651820977572ArticleGoogle Scholar
- Chakravarty A, Mahanta C (2016) Actuator fault-tolerant control (FTC) design with post-fault transient improvement for application to aircraft control. Int J Robust Nonlin 26:2049–2074. https://doi.org/10.1002/rnc.3392ArticleMathSciNetGoogle Scholar
- Tiwari PM, Janardhanan S, Nabi MU (2016) Attitude control using higher order sliding mode. Aerosp Sci Technol 54:108–113. https://doi.org/10.1016/j.ast.2016.04.012ArticleGoogle Scholar
- Van H, Dinh BH (2016) Second order sliding mode control design for active magnetic bearing system. AETA 2015: Recent Adv Electr Eng Relat Sci 371:519–529. https://doi.org/10.1007/978-3-319-27247-4_44ArticleGoogle Scholar
- Kong M, Yang M, Li RZ, Long YZ, Zhang J, Huang X, Cui X, Zhang YB, Said Z, Li CH (2023) Graphene-based flexible wearable sensors: mechanisms, challenges, and future directions. Int J Adv Manuf Technol. https://doi.org/10.1007/s00170-023-12007-7ArticleGoogle Scholar
- Boiko IM (2013) Chattering in sliding mode control systems with boundary layer approximation of discontinuous control. Int J Syst Sci 44:1126–1133. https://doi.org/10.1080/00207721.2011.652233ArticleMathSciNetGoogle Scholar
- Jain S, Mishra JP, Talange DB (2015) A robust control approach for magnetic levitation system based on super-twisting algorithm. In: 2015 10th Asian Control Conference (ASCC) pp 1–6. https://doi.org/10.1109/ASCC.2015.7244600
- Kandil MS, Micheau P, Trovão JP, Bakay LS, Dubois MR (2015) Hybrid Magnetic Bearing Regulation via Super Twisting Control. In: 2015 15th International Conference on Control, Automation and Systems (ICCAS), pp 1566–1571. https://doi.org/10.1109/ICCAS.2015.7364606
- Chen Q, Liu G, Zheng SQ (2015) Suppression of imbalance vibration for AMBs controlled driveline system using double-loop structure. J Sound Vib 337:1–13. https://doi.org/10.1016/j.jsv.2014.09.047ArticleGoogle Scholar
- Tonoli A, Bonfitto A, Silvagni M, Suarez LD, Beltran-Carbajal F (2012) Rotors on active magnetic bearings: modeling and control techniques. Adv Vib Eng Struct Dyn 2012:1–3. https://doi.org/10.5772/51298
- Dominguez JR, Mora-Soto C, Ortega-Cisneros S, Panduro JJR, Loukianov AG (2012) Copper and core loss minimization for induction motors using high-order sliding-mode control. IEEE T Ind Electron 59:2877–2889. https://doi.org/10.1109/tie.2011.2171170ArticleGoogle Scholar
- Levant A (1993) Sliding order and sliding accuracy in sliding mode control. Int J Control 58:1247–1263. https://doi.org/10.1080/00207179308923053ArticleMathSciNetGoogle Scholar
- Kang MS, Lyou J, Lee JK (2010) Sliding mode control for an active magnetic bearing system subject to base motion. Mechatronics 20:171–178. https://doi.org/10.1016/j.mechatronics.2009.09.010ArticleGoogle Scholar
- Utkin V (2016) Discussion aspects of high-order sliding mode control. IEEE T Automat Contr 61:829–833. https://doi.org/10.1109/tac.2015.2450571ArticleMathSciNetGoogle Scholar
- Reichhartinger M, Horn M (2009) Application of higher order sliding-mode concepts to a throttle actuator for gasoline engines. IEEE T Ind Electron 56:3322–3329. https://doi.org/10.1109/tie.2009.2026382ArticleGoogle Scholar
- Plestan F, Shtessel Y, Bregeault V, Poznyak A (2010) New methodologies for adaptive sliding mode control. Int J Control 83:1907–1919. https://doi.org/10.1080/00207179.2010.501385ArticleMathSciNetGoogle Scholar
- Liu Z, Bi C, Jiang Q, Wu D (2005) Automatic learning control for unbalance compensation in active magnetic bearings. IEEE Trans Magn 41:2270–2280. https://doi.org/10.1109/tmag.2005.851866ArticleGoogle Scholar
- Pisano A, Davila A, Fridman L, Usai E (2008) Cascade control of PM DC drives via second-order sliding-mode technique. IEEE T Ind Electron 55:3846–3854. https://doi.org/10.1109/tie.2008.2002715ArticleGoogle Scholar
- Wang XY, Zhang YP, Gao P (2020) Design and analysis of second-order sliding mode controller for active magnetic bearing. Energies 13:5965. https://doi.org/10.3390/en13225965ArticleGoogle Scholar
- Kandil MS, Dubois MR, Bakay LS, Trovao JPF (2018) Application of second-order sliding-mode concepts to active magnetic bearings. IEEE T Ind Electron 65:855–864. https://doi.org/10.1109/tie.2017.2721879ArticleGoogle Scholar
- Saha S, Amrr SM, Saidi AS, Banerjee A, Nabi M (2021) Finite-time adaptive higher-order SMC for the nonlinear five DOF active magnetic bearing system. Electronics 10:1333. https://doi.org/10.3390/electronics10111333
- Yu X, Zhihong M (1998) Multi-input uncertain linear systems with terminal sliding-mode control. Automatica 34:389–392. https://doi.org/10.1016/s0005-1098(97)00205-7ArticleMathSciNetGoogle Scholar
- Xu WJ, Qu SC, Zhang C (2023) Fast terminal sliding mode current control with adaptive extended state disturbance observer for PMSM system. IEEE J Em Sel Top P 11:418–431. https://doi.org/10.1109/jestpe.2022.3185777ArticleGoogle Scholar
- Liu HC, Cao Z, Kang HM, Ouyang ZH, Duan LH (2023) Adaptive backstepping dynamic sliding mode control for maglev turning motor spindle based on limited time preset performance. J Vib Control. https://doi.org/10.1177/10775463231185482ArticleGoogle Scholar
- Xu B, Zhu H, Ji W (2013) Adaptive nonsingular terminal sliding model control of the suspension system for a bearingless permanent magnet synchronous motor. In: Proceedings of the 32nd Chinese Control Conference 2013, pp 4322–4326.
- Zhao YT, Wei C, Wei QS, Zhao Y (2022) High precision control of rotating payload satellite considering static and dynamic unbalanced disturbance. Int J Aerospace Eng 2022:8281092. https://doi.org/10.1155/2022/8281092ArticleGoogle Scholar
- Abooee A, Arefi MM (2019) Robust finite-time stabilizers for five-degree-of-freedom active magnetic bearing system. J Franklin I 356:80–102. https://doi.org/10.1016/j.jfranklin.2018.08.026ArticleMathSciNetGoogle Scholar
- Bai XF, Jiang J, Li CH, Dong L, Ali HM, Sharma S (2023) Tribological performance of different concentrations of Al2O3 nanofluids on minimum quantity lubrication milling. Chin J Mech Eng 36:11. https://doi.org/10.1186/s10033-022-00830-0ArticleCASGoogle Scholar
- Yang ZB, Zhang D, Sun XD, Sun WM, Chen L (2017) Nonsingular fast terminal sliding mode control for a bearingless induction motor. IEEE Access 5:16656–16664. https://doi.org/10.1109/access.2017.2739199ArticleGoogle Scholar
- Yu YJ, Yang ZH, Han C, Liu H (2018) Fuzzy adaptive back-stepping sliding mode controller for high-precision deflection control of the magnetically suspended momentum wheel. IEEE T Ind Electron 65:3530–3538. https://doi.org/10.1109/tie.2017.2750617ArticleGoogle Scholar
- Giap VN, Huang SC (2020) Effectiveness of fuzzy sliding mode control boundary layer based on uncertainty and disturbance compensator on suspension active magnetic bearing system. Meas Control 53:934–942. https://doi.org/10.1177/0020294020905044ArticleGoogle Scholar
- Yao X, Chen ZB, Mao YH (2019) A dual-loop control approach of active magnetic bearing system for rotor tracking control. IEEE Access 7:121760–121768. https://doi.org/10.1109/access.2019.2937191ArticleGoogle Scholar
- Yao X, Chen ZB (2019) Sliding mode control with deep learning method for rotor trajectory control of active magnetic bearing system. T I Meas Control 41:1383–1394. https://doi.org/10.1177/0142331218778324ArticleGoogle Scholar
- Cui G, Wang N, Su B, Cai B, Chu X (2019) Research on suspension control based on model mismatch compensator for Maglev Yaw system of wind turbines. In: 2019 Chinese Automation Congress (CAC), pp 4953-4958. https://doi.org/10.1109/CAC48633.2019.8997366
- Han JQ (2009) From PID to active disturbance rejection control. IEEE T Ind Electron 56:900–906. https://doi.org/10.1109/tie.2008.2011621ArticleGoogle Scholar
- Huang Y, Cai H, Bai X (2017) Research on stability control of floated inertial platform based on ADRC. Int Conf Optoelectron Microelectron Technol Appl 10244:102440M. https://doi.org/10.1117/12.2261957ArticleGoogle Scholar
- Ji J, Hansen C (2001) Non-linear oscillations of a rotor in active magnetic bearings. J Sound Vib 240:599–612. https://doi.org/10.1006/jsvi.2000.3257ArticleGoogle Scholar
- Jin CW, Guo KX, Xu YP, Cui HB, Xu LX (2019) Design of magnetic bearing control system based on active disturbance rejection theory. J Vib Acoust 141:011009. https://doi.org/10.1115/1.4040837
- Han X, Liu G, Chen BD, Zheng SQ (2022) Surge disturbance suppression of AMB-rotor systems in magnetically suspension centrifugal compressors. IEEE Trans Control Syst Technol 30:1550–1560. https://doi.org/10.1109/tcst.2021.3112765ArticleGoogle Scholar
- Shi Y-L, Hou C-Z (2008) Design of improved nonlinear tracking differentiator. Control Decis 23:647–650. https://doi.org/10.1038/cgt.2008.5ArticleMathSciNetCASGoogle Scholar
- Yu TT, Zhang ZZ, Li Y, Zhao WL, Zhang JC (2023) Improved active disturbance rejection controller for rotor system of magnetic levitation turbomachinery. Electron Res Arch 31:1570–1586. https://doi.org/10.3934/era.2023080ArticleGoogle Scholar
- Zhu HQ, Gu ZW (2020) Active disturbance rejection control of 5-degree-of-freedom bearingless permanent magnet synchronous motor based on fuzzy neural network inverse system. ISA Trans 101:295–308. https://doi.org/10.1016/j.isatra.2020.01.028ArticlePubMedGoogle Scholar
- Xu YH, Wang XY, Liu MX, Li N, Yu T (2023) Adaptive robust control of active magnetic bearings rigid rotor systems. J Power Electron 23:1004–1015. https://doi.org/10.1007/s43236-022-00578-9ArticleGoogle Scholar
- Steyn SJM, Van Vuuren P, Van Schoor G (2010) Multivariable H∞ control for an active magnetic bearing flywheel system. UKACC Int Conf Control 2010:1014–1019. https://doi.org/10.1049/ic.2010.0420ArticleGoogle Scholar
- Kuseyri İS (2012) Robust control and unbalance compensation of rotor/active magnetic bearing systems. J Vib Control 18:817–832. https://doi.org/10.1177/1077546310397560ArticleMathSciNetGoogle Scholar
- Jastrzebski RP, Hynynen KM, Smirnov A (2010) H(infinity) control of active magnetic suspension. Mech Syst Signal Pr 24:995–1006. https://doi.org/10.1016/j.ymssp.2009.10.008ArticleGoogle Scholar
- Zhu C, Zhong Z (2015) H∞ robust controller design and experimental analysis of active magnetic bearings with flexible rotor system. Int J Eng 28:1233–1240. https://doi.org/10.5829/idosi.ije.2015.28.08b.17ArticleGoogle Scholar
- Wang M, Cole MOT, Keogh PS (2017) New LMI based gain-scheduling control for recovering contact free operation of a magnetically levitated rotor. Mech Syst Signal Pr 96:104–124. https://doi.org/10.1016/j.ymssp.2017.04.008ArticleCASGoogle Scholar
- Lusty C, Keogh P (2018) Active vibration control of a flexible rotor by flexibly mounted internal-stator magnetic actuators. IEEE-ASME T Mech 23:2870–2880. https://doi.org/10.1109/tmech.2018.2869023ArticleGoogle Scholar
- Noshadi A, Zolfagharian A (2019) Unbalance and harmonic disturbance attenuation of a flexible shaft with active magnetic bearings. Mech Syst Signal Pr 129:614–628. https://doi.org/10.1016/j.ymssp.2019.04.055ArticleGoogle Scholar
- Maslen EH, Sawicki JT (2007) Mu-synthesis for magnetic bearings: why use such a complicated tool? ASME Int Mech Eng Congr Exposition 43033:1103–1112. https://doi.org/10.1115/IMECE2007-43910ArticleGoogle Scholar
- Ran SL, Hu YF, Wu HC, Cheng X (2018) Resonance vibration control for AMB flexible rotor system based on mu-synthesis controller. Math Probl Eng 2018:1–16. https://doi.org/10.1155/2018/4362101ArticleGoogle Scholar
- Mushi SE, Lin Z, Allaire PE (2010) Stability analysis for a flexible rotor on active magnetic bearings subject to aerodynamic loads. In; Proceedings of the 12th International Symposium on Magnetic Bearings, Wuhan, China, pp 21–24
- Pesch AH, Hanawalt SP, Sawicki JT (2014) A case study in control methods for active magnetic bearings. Dyn Syst Control Conf 46186:V001T012A003. https://doi.org/10.1115/DSCC2014-6257ArticleGoogle Scholar
- Riemann B, Sehr MA, Schittenhelm RS, Rinderknecht S (2013) Robust control of flexible high-speed rotors via mixed uncertainties. In: 2013 European Control Conference (ECC), pp 2343–2350. https://doi.org/10.23919/ECC.2013.6669786
- Mystkowski A (2010) Sensitivity and stability analysis of mu-synthesis AMB flexible rotor. Solid State Phenom 164:313–318. https://doi.org/10.4028/www.scientific.net/SSP.164.313ArticleCASGoogle Scholar
- Hu YF, Yang KZ, Wu HC, Guo XH, Wang NX (2022) Critical vibration and control of the Maglev high-speed motor based on mu-synthesis control. Sensors 22:8692. https://doi.org/10.3390/s22228692ArticlePubMedPubMed CentralGoogle Scholar
- Ma X, Zheng SQ, Wang K (2019) Active surge control for magnetically suspended centrifugal compressors using a variable equilibrium point approach. IEEE T Ind Electron 66:9383–9393. https://doi.org/10.1109/tie.2019.2891412ArticleGoogle Scholar
- Rawlings JB, Mayne DQ, Diehl M (2017) Model predictive control: theory, computation, and design, vol 2. Nob Hill Publishing Madison, WI: Nob Hill Publishing
- Bavili RE, Khosrowjerdi MJ, Vatankhah R (2015) Active fault tolerant controller design using model predictive control. Control Eng Appl Inf 17:68–76 Google Scholar
- Tsai Y-W, Van Duc P, Duong VA, Trang NC, Chu TD (2016) Model predictive control nonlinear system of active magnetic bearings for a flywheel energy storage system. In: AETA 2015: Recent Advances in Electrical Engineering and Related Sciences, pp 541–551. https://doi.org/10.1007/978-3-319-27247-46
- Morsi A, Abbas HS, Ahmed SM, Mohamed AM (2022) Imbalance compensation of active magnetic bearing systems using model predictive control based on linear parameter-varying models. J Vib Control. https://doi.org/10.1177/10775463221099074ArticleGoogle Scholar
- Bachle T, Hentzelt S, Graichen K (2013) Nonlinear model predictive control of a magnetic levitation system. Control Eng Pract 21:1250–1258. https://doi.org/10.1016/j.conengprac.2013.04.009ArticleGoogle Scholar
- Molina LMC, Bonfitto A, Galluzzi R (2021) Offset-free model predictive control for a cone-shaped active magnetic bearing system. Mechatronics 78:102612. https://doi.org/10.1016/j.mechatronics.2021.102612
- Morsi A, Abbas HS, Ahmed SM, Mohamed AM (2021) Model predictive control based on linear parameter-varying models of active magnetic bearing systems. IEEE Access 9:23633–23647. https://doi.org/10.1109/access.2021.3056323ArticleGoogle Scholar
- Qi W, Liu J, Chen X (2011) Supervisory predictive control of standalone wind/solar energy generation systems. IEEE Trans Control Syst Technol 19:199–207. https://doi.org/10.1109/tcst.2010.2041930ArticleGoogle Scholar
Funding
This study was financially supported by the National Key Research and Development Program, China (2020YFB2010500), the National Natural Science Foundation of China (52105457,51975305), the Special Fund of Taishan Scholars Project (tsqn202211179), the Youth Talent Promotion Project in Shandong (SDAST2021qt12), and the Natural Science Foundation of Shandong Province (ZR2023QE057, ZR2022QE028, ZR2021QE116, and ZR2020KE027).